Next: Model of Hipparchus
Up: Geometric Planetary Orbit Models
Previous: Introduction
Kepler's geometric model of a heliocentric planetary orbit is summed up in his three well-known laws of planetary motion.
According to Kepler's first law, all planetary orbits are ellipses which are confocal with the sun and lie in a
fixed plane.
Moreover, according to Kepler's second law, the radius vector which connects the sun to a given planet sweeps out equal areas in equal time intervals.
Figure 17:
A Keplerian orbit.
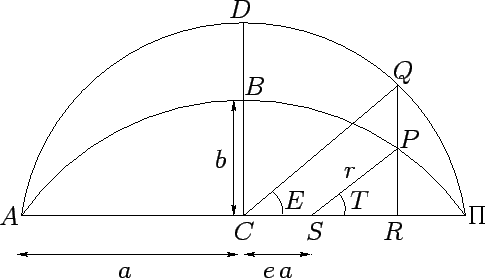 |
Consider Figure 17.
is half of an elliptical planetary orbit. Furthermore,
is the
geometric center of the orbit,
the focus at which the sun is located,
the instantaneous position of the planet,
the perihelion point (i.e., the planet's point of closest approach to the sun),
and
the aphelion point (i.e., the point of furthest distance from the sun). The ellipse is symmetric about
, which is termed the major axis, and about
,
which is termed the minor axis.
The length
is called the orbital
major radius. The length
represents the displacement of the sun from
the geometric center of the orbit, and is generally written
, where
is termed the
orbital eccentricity, where
. The length
is called
the orbital minor radius.
The length
represents the radial distance of the planet from the sun.
Finally, the angle
is the angular bearing of the planet from the sun,
relative to the major axis of the orbit, and is termed the true anomaly.
is half of a circle whose geometric center is
, and whose
radius is
. Hence, the circle passes through the perihelion and aphelion
points.
is the point at which the perpendicular from
meets the major axis
. The point where
produced
meets circle
is denoted
. Finally,
the angle
is called the elliptic anomaly.
Now, the equation of the ellipse
is
 |
(60) |
where
and
are the perpendicular distances from the minor and major
axes, respectively. Likewise, the equation of the circle
is
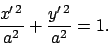 |
(61) |
Hence, if
then
 |
(62) |
and it follows that
 |
(63) |
Now,
. Furthermore, it is easily demonstrated that
,
,
, and
. Consequently, Eq. (63) yields
 |
(64) |
Also, since
, we have
 |
(65) |
Taking the square root of the sum of the squares of the previous two equations, we obtain
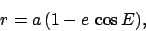 |
(66) |
which can be combined with Eq. (65) to give
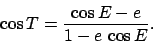 |
(67) |
Now, according to Kepler's second law,
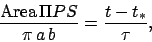 |
(68) |
where
is the time at which the planet passes point
,
the time at which it passes the perihelion point,
and
the orbital period.
However,
But,
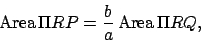 |
(70) |
since
for all values of
. In addition,
 |
|
|
(71) |
Hence, we can write
 |
(72) |
According to Eqs. (64) and (65),
, and
, so
the above expression reduces to
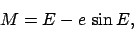 |
(73) |
where
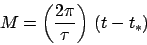 |
(74) |
is an angle which is zero at the perihelion point, increases uniformly in time, and has a repetition period which
matches the period of the planetary orbit. This angle is termed the mean anomaly.
In summary, the radial and angular polar coordinates,
and
, respectively,
of a planet in a Keplerian orbit about the sun are specified as implicit functions
of the mean anomaly, which is a linear function of time, by the
following three equations:
It turns out that the earth and the five visible planets all possess low eccentricity orbits characterized by
. Hence, it is a good approximation to expand the above three equations
using
as a small parameter. To second-order, we
get
Finally, these equations can be combined to give
and
as explicit functions of the
mean anomaly:
Next: Model of Hipparchus
Up: Geometric Planetary Orbit Models
Previous: Introduction
Richard Fitzpatrick
2010-07-21